An off-grid solar system consists of batteries, solar panels, and charge controllers. Of these components, the battery is the most expensive, making it even more important to care for throughout its lifetime. Infrastructure owners require batteries to last for their specified lifetime in order to achieve expected returns on investment, and households need healthy batteries in order to retain power throughout the night and maximize grid uptime. However, it proves difficult to define battery health, let alone measure it. Nonetheless, we have set out to do just that because as we continue to scale, maintaining the long-term health of our batteries is crucial.
Battery health is defined as the battery’s energy capacity
But what does it mean for a battery to be healthy? Typically, a battery’s state of health is defined by its energy capacity, meaning the amount of energy that a battery can deliver from 100% state of charge (SoC) to 0%. This capacity value is usually given as the amount of current that can be drawn from the battery over time in Ampere hours (Ah). For example, a 50 Ah capacity battery can deliver a current of 50 Amps for 1 hour, or 25 Amps for 2 hours, and so on (at least in theory!).
As batteries age, their capacity decreases. For the purposes of our analysis, we consider a battery to be degraded when its capacity decreases below 70% of its initial capacity. Therefore, a battery’s life span is defined as the amount of time it takes for the capacity to reach 70% of its original value.
Our off-grid mesh-grid networks demand high performance & durable batteries
In Okra’s mesh-grids, batteries are charged and discharged on a daily basis. Although energy consumption varies between households, the batteries typically see frequent usage throughout the day and night. Additionally, households with a surplus of energy can share power with other households that need it, making it very important for us to efficiently capture all of the energy available at an individual household.
Because these batteries are used daily and are expected to last for more than just a couple of years, lithium-ion battery chemistries are the clear choice over lead-acid batteries. Whilst lithium-ion batteries are more expensive upfront compared to their lead-acid counterparts, they offer significant advantages in energy density, charge efficiency, and life span, making them well worth the initial investment. Specifically, we will be discussing lithium iron phosphate (LiFePo) batteries, as this battery chemistry is a good fit for the demands of off-grid energy storage.
Batteries degrade through calendar aging & cycle aging
There are two distinct aging modes that contribute to the degradation of a battery over time: calendar aging and cycle aging.
As the name suggests, calendar aging consists of all the aging processes that contribute to battery cell aging aside from the usage of the battery
In order to consider this effect in isolation, it is helpful to ask: if a battery is left in storage, how quickly will it lose capacity? However, calendar aging is not strictly limited to storage cases. Calendar aging can also be the primary mode of aging in cases where batteries are at rest for a vast majority of their life span, such as an electric vehicle that is only driven for 30 minutes each day.
In contrast, cycle aging is the result of charging and discharging the battery repeatedly. Although there is no standard definition of a single discharge cycle, manufacturers often assume 80% depth-of-discharge (DoD) when giving their ratings. DoD is defined as the per cent of battery capacity used in a single discharge cycle. For example, discharging from 100% SoC to 20%, and finally charging back to 100% constitutes a single cycle at 80% DoD.
Because of these two distinct processes, battery manufacturers often specify the expected life span of their batteries by providing both the number of years and number of cycles that the battery should last. For example, a battery may be rated for a life span of 5 years / 5000 cycles, whichever comes first.
How much of an impact does calendar aging have on our batteries?
Since our batteries are being charged and discharged every single day, calendar aging should only make up a small proportion of the total aging effects. Nevertheless, it is important to understand the extent of calendar aging’s effect as a baseline.
There are two main factors that contribute to calendar aging: temperature and SoC. Both a higher temperature and a higher full charge SoC increase the rate of aging. While we cannot control the temperature, we do have the ability to control the full charge SoC of our batteries. Reducing this threshold, however, would mean restricting the available power in the network, but luckily, LFP cells are more resilient to storage at high SoC values than other lithium-ion cells.
Let’s examine the calendar aging effects for LFP cells stored at 100% SoC over a range of temperatures (credit to Catton).
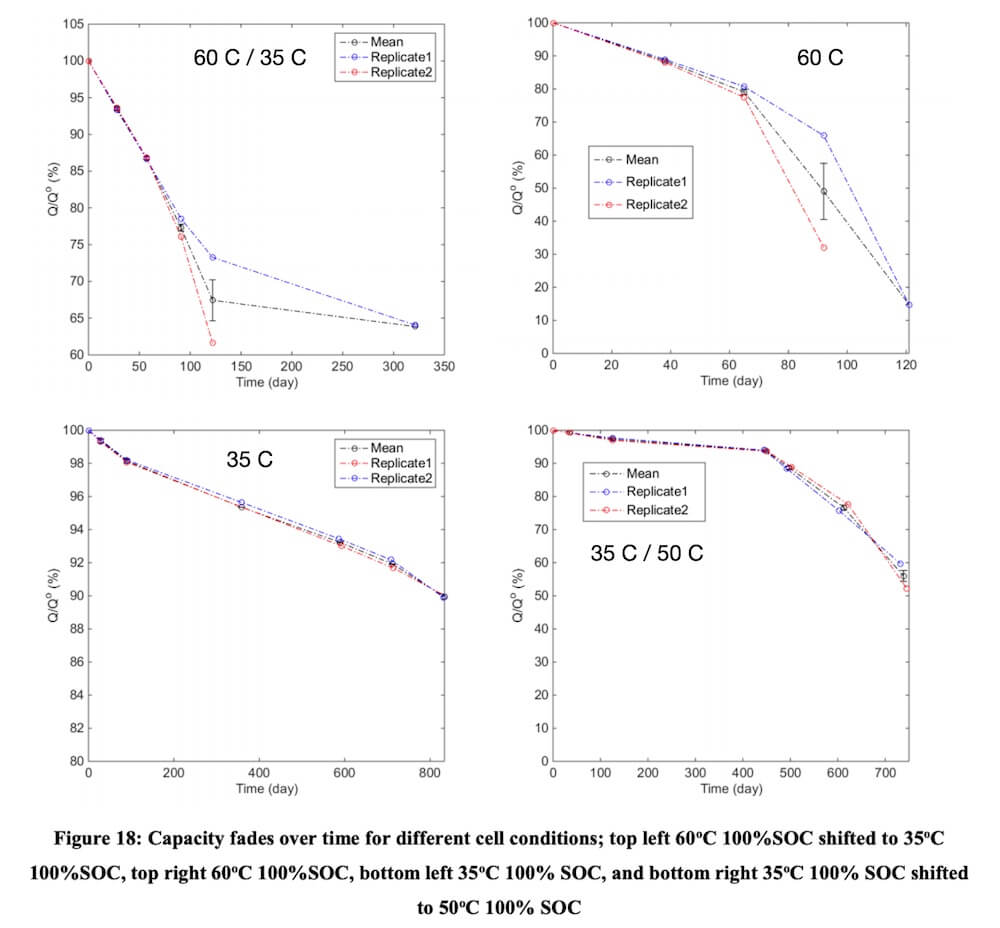
We can focus our attention on the plot in the bottom left, as a temperature of 35 C is closest to the temperatures that our batteries will see in the field. Even at this constant temperature of 35 C and SoC of 100%, the cell only loses roughly 10% of its capacity over the duration of the experiment. While this would be concerning if extrapolated in a linear fashion – a 30% loss in capacity over 7 years due to calendar aging alone – there are actually several reasons to be optimistic.
First, calendar aging does not continue to progress in a linear fashion. Beltran et. al. [2] points out that the aging curve is closer to a logarithmic progression, and in fact, the calendar aging observed in the first 800 days may only double itself after another nearly 3000 day. Moreover, some of the calendar aging losses are actually recoverable when the battery is once again cycled regularly.
Second, many of our batteries will only be in standby mode for a small proportion of their life span. During the day, they are often being charged by solar or discharged for household consumption and at night, the home (or neighbouring homes) may still use the battery for various low power applications. Even if it is at rest during the night, the battery’s SoC will be lower at this point, significantly slowing the aging effects.
And finally, the temperatures will be slightly lower in the field. The batteries are most likely to be on standby at night, when the temperatures are lower than 35 C, further reducing the rate of calendar aging.
The combination of these factors means that calendar aging should end up contributing relatively little to the total aging of our batteries. The dominant contribution will come from cycle aging.
How can we mitigate the effects of cycle aging?
When it comes to cycle aging, the two most important variables are temperature and DoD. Both a higher DoD and temperature increase the rate of cycle aging.
In this comprehensive review, Spitthoff et. al. summarize the literature’s findings regarding the impact of several key variables on the aging rate of different types of lithium-ion cells. We can explore the data gathered on temperature and DoD to learn how we expect the life span of our batteries to be affected. Let us first examine the effect of temperature on cycle aging. The figure below shows the normalized effect of temperature on the aging rate for different types of lithium-ion cells.
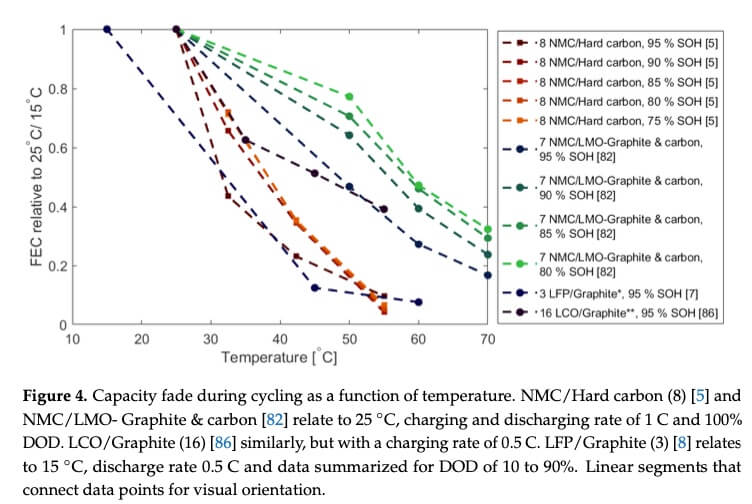
The figure is dense but the most important takeaway is that the y-axis is plotting the relative life span of the battery cells. So for example, if the LFP cell in the figure (the one that starts furthest to the left) can withstand 10,000 full cycles at 15 C, then we would expect it to hold up to around 5,000 full cycles at 30 C (since the y-value at this temperature is approximately 0.5).
While the LFP cell has one of the longest life spans (shown below), it appears to be more sensitive to temperature increases than other lithium-ion cells. This is important to consider if we need to adjust cycle life spans from ratings that are given at 20 or 25 C, and it highlights the importance of keeping the batteries in a shaded and cool place as much as possible in the field.
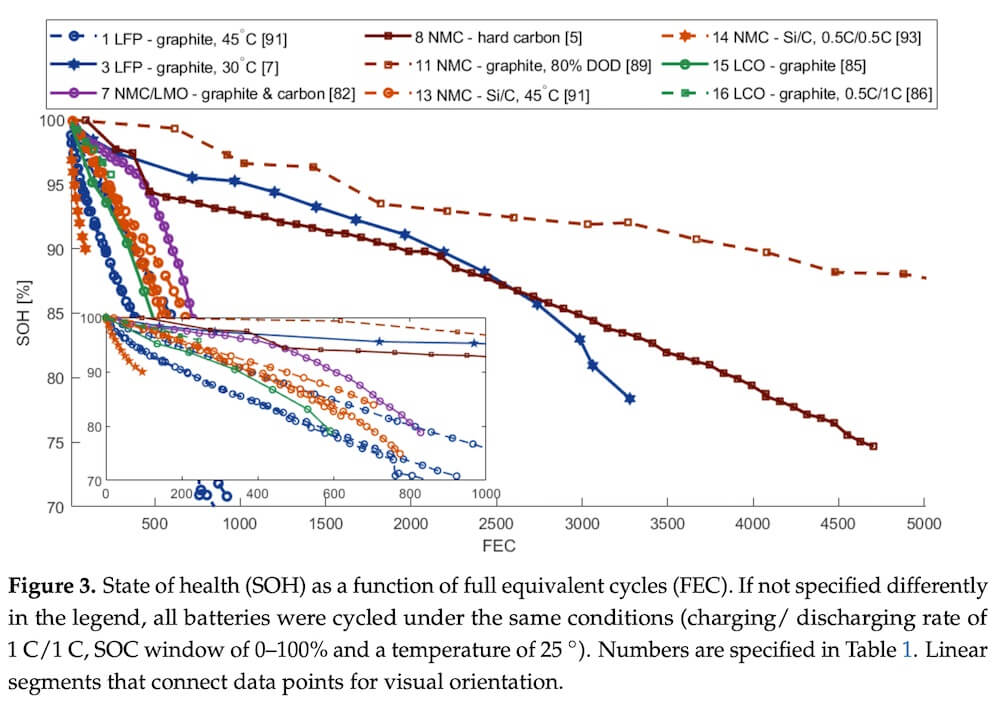
Now, we can tackle the effect of DoD on cycle aging. The same paper provides the following figure illustrating the impact of DoD on cycle life span.
As noted in the below figure, DoD’s are typically referenced around the 50% SoC mark (meaning that a 60% DoD would run from 20% – 80% SoC) because time spent in the high and low SoC regions can unnecessarily stress the battery.
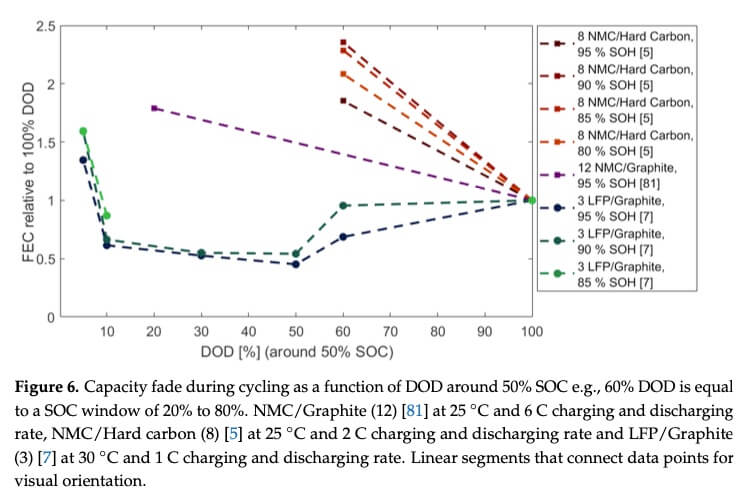
While other battery types show a clear trend – the life span decreases as DoD increases – the results differ for LFP as there are two different plateau regions and no obvious trend can be seen. This could be explained by the fact that the voltage window for an LFP cell is much more narrow than for other lithium-ion cells, and its voltage never approaches the max voltage reached by NMC and LCO cells. Thus, it could be that reducing the usable SoC window may not actually relieve significant stress from the cell.
On the other hand, there are multiple sources in the literature such as Groot and Wikner suggesting that DoD does play a significant role in the life span of LFP cells. These findings conflict with the literature review, so we hypothesize that DoD does play a role in the aging of LFP cells, but likely with a reduced effect compared to other lithium-ion cells. However, it is difficult to specify the magnitude of this effect due to the disagreement in the literature.
Using this working hypothesis then, we choose to use a DoD of 80%, which has become somewhat of a default value specified by manufacturers when rating the cycle life span of their batteries. This allows us to be confident in the life span ratings provided by manufacturers, knowing that we are treating our batteries at least as well as they intend, if not better. Furthermore, this provides the added practical benefit of avoiding situations where the battery’s voltage tanks (perhaps due to slightly unbalanced cells, or just extremely low SoC) as its SoC dissipates, requiring manual intervention to charge the battery directly before it can be reconnected to the system.
We’ll be keeping a close eye on our batteries
As we’ve seen, the literature on battery health can be confusing and even conflicting at times, and the state of battery research continues to evolve over the years.
In an effort to combat some of this uncertainty, we are building on our state of charge work to produce a measure of battery state of health, allowing us to track battery health more accurately than we ever could in the past.
Going forward, we will monitor this data closely and use it to tune our battery management algorithms as well as validate the specifications of our batteries